Imagine a computer that could crack the encryption that currently safeguards all digital information from financial transactions to your personal identity — essentially breaking the internet as we know it. Now imagine this same computer could solve complex problems in a matter of minutes — problems that would take a classical computer many years to figure out. This kind of processing power could transform how the world addresses issues like climate change, drug development, national security and food scarcity.
This computer is a quantum computer, a machine that would revolutionize what computers are capable of doing—and scientists and engineers around the world are racing to develop the first full-scale quantum computer to unlock this potential.
But, the immense potential of a quantum computer is blocked by the immense challenge of developing the technology and science behind it. Quantum computing is more complex than classical computing because of the depth of the foundational science that underlies it. And, there’s still a lot of scientific work to be done before the world can go quantum.
Which is where University of Wisconsin–Madison researchers come in.
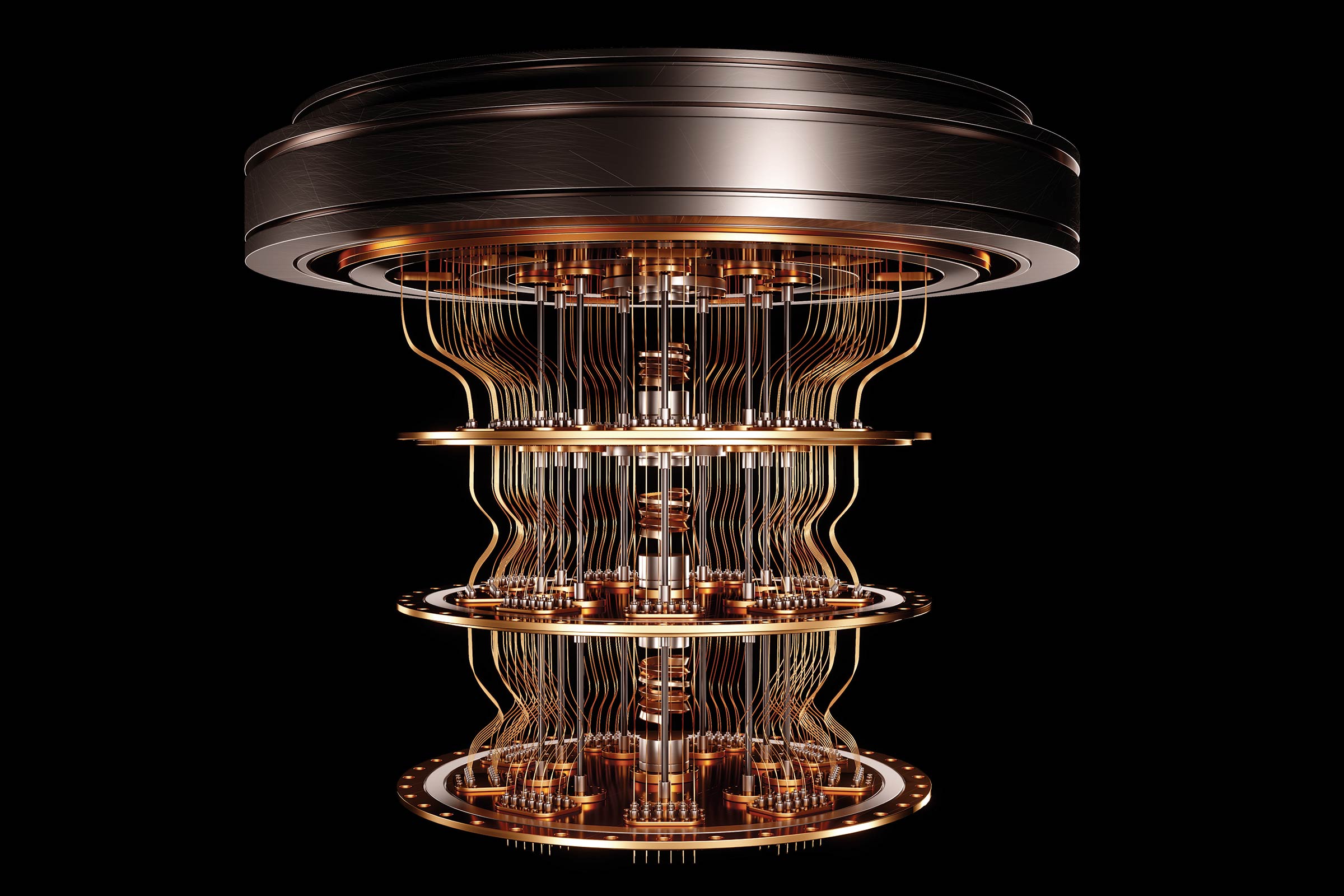
Researchers like Mark Eriksson, John Bardeen Professor of Physics and chair of the physics department. Eriksson has been working in quantum physics since 2001, tackling the underlying technology that could propel quantum computing. Eriksson’s research focuses on exploring one of the more promising approaches to building a quantum computer.
One of the reasons it’s so hard to develop quantum computers is that there’s no clear and superior approach to building these machines, he explains. It’s unlike the approach to building classical computers, which is well established and used by every major technology company.
“We don’t know which approaches will be best for which parts of a quantum
computer, or if there’s one approach that will be ideal for all parts of a quantum computer,” he says. “The reason it’s so hard to figure this out is that there are features of quantum computers that are incredibly challenging to develop.”
One key difference between classical and quantum computers is that, at the root of this challenge, today’s digital technology uses the language of bits, which consists of binary signals coded with the value of a zero or one. This language powers everything from a Zoom call to a Google search, to streaming your favorite Netflix show.
The reason it’s so hard to figure this out is that there are features of quantum computers that are incredibly challenging to develop.
But in quantum computing, the bits are replaced by quantum bits, or “qubits,” which can be not just either zeros or ones but can be both at the same time. This characteristic is known as “superposition,” and it’s one of the reasons quantum computing has so much potential—there are exponentially more value combinations when you link qubits together.
Harnessing that superposition quality is one of the key challenges of building a quantum computer, due to a core principle of quantum mechanics.
“In quantum mechanics, there’s a fundamental notion that when you look at something or try to measure something, you disturb it,” explained Mark Saffman, Johannes Rydberg Professor of Physics, who has also been working in quantum physics since 2001.
“If you try to look at, or measure, a quantum particle, doing so will push it and change it.”
Picture a coin spinning and landing on a table. If that coin was a qubit and you looked at it after it landed, you would erase its superposition power to be either a zero or a one—and fix its value instead—which would eliminate one of its most promising qualities for quantum computing.
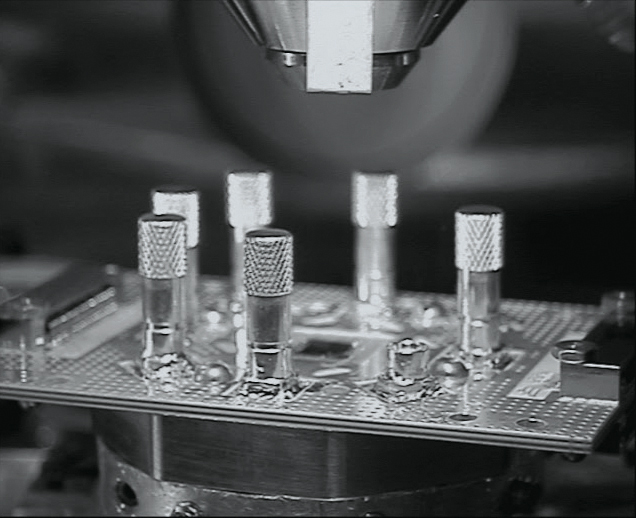
“This characteristic of qubits offers huge potential for writing algorithms that will power quantum computing,” says Eriksson. “But it’s a very fragile state— and one of the greatest challenges of quantum computing is finding a way to maintain a qubit’s quantum state.”
Tackling this challenge is a main pillar of Eriksson’s work to develop the foundational technology that could enable quantum computers.
Eriksson uses silicon to make qubits and leverages nanoscale electronic systems to manipulate them. Silicon is used in classical computing, and he says that it offers the advantage of being able to apply some of the same materials and technology to a quantum approach.
Eriksson traps electrons in the silicon using tiny metal gates, much like in a classical computer chip, and turns the electrons into qubits. The small metal gates allow him to contain and control the electrons, which he can move around through a high frequency voltage. He uses a magnetic force to assign and flip the values of the qubits between one and zero, similar to how magnetic force can be used to rotate a bar magnet between a north and a south pole.
This field has really evolved beyond physics because we’re now at the stage where we know that the fundamental principles and ideas are valid, but we need to actually build full-scale quantum computers.
This is all done on a nanoscale level, which means he’s working with a scale that measures materials by the billionth of a meter. He uses cutting-edge nanofabrication technology to do this work through UW’s Nanoscale Fabrication Center.
Another key feature—and challenge—of quantum computing is quantum entanglement, which means that qubits can be manipulated to change values depending on the value of other qubits. This feature is what will enable quantum computers to run the really powerful algorithms that will elevate computing to the next level. But, entanglement is tricky due to the fragility of a qubit’s superposition—you need to be able to change the value of qubits without looking at them or measuring them.
“It’s really important to isolate qubits from anything that might change their superposition,” Eriksson explains. “But, we need to create strong interactions between qubits, because that’s what powers useful algorithms.”
Eriksson’s lab has done this on a small scale by placing the qubits 40 nanometers apart, which is so close that it’s nearly impossible to comprehend just how tightly packed together they are. This proximity allows Eriksson to create strong interactions between qubits and entangle them. He has seen promising results through this method, but scaling it up is another challenge.
“We still have a long road ahead of us,” he says.
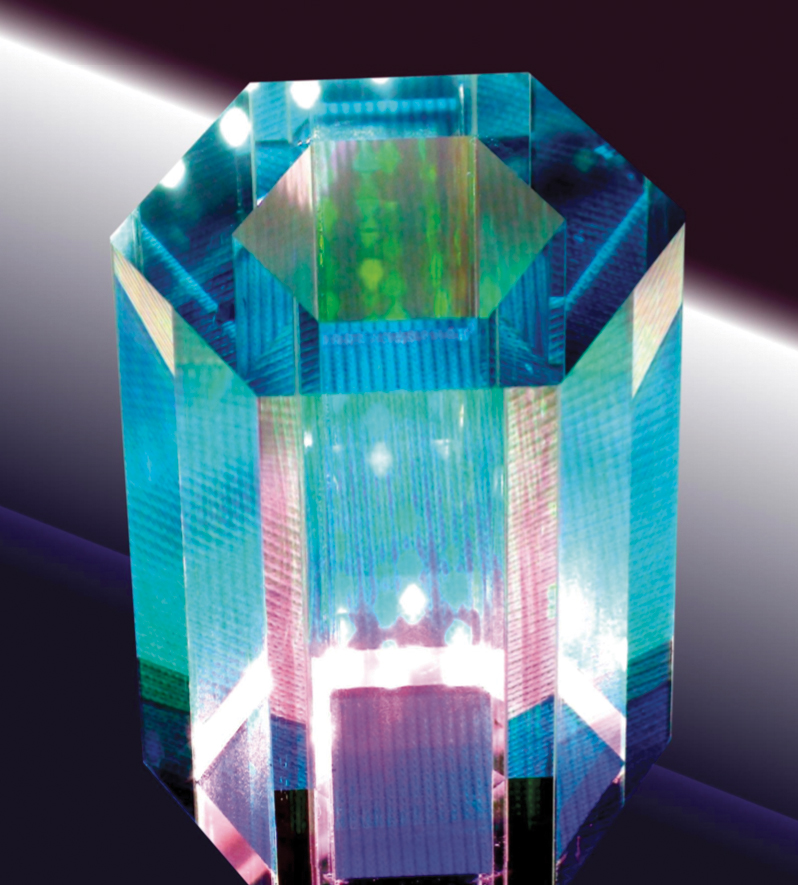
A usable quantum computer is years away by all accounts, but Eriksson’s research trajectory offers a compelling example of how much this technology has progressed over the last two decades.
“When I started in 2001, we couldn’t even contain one electron in silicon—never mind turning it into a qubit,” he says.
Silicon qubits are one of the major kinds of qubits driving the conversation around quantum technology, and researchers at UW–Madison cover nearly the entire spectrum of relevant qubits. It’s one of the many examples of the institutional strength in quantum computing at UW, says Eriksson.
Saffman works with atomic qubits, another one of the most promising types, and says that one of the values of turning atoms into qubits is that they’re “nature’s qubits.”
Atoms are readily available and don’t need to be fabricated, he explains. Atoms also have a natural uniformity, unlike fabricated qubits, which can have more irregularities, so atoms are a more reliable material to work with.
To turn atoms into qubits, Saffman isolates the atoms in a vacuum chamber and uses lasers to chill them so that they will remain stationary and isolated. Another series of lasers traps them so that they can be controlled and worked with, and yet another set of lasers controls the qubits and entangles them.
Saffman’s lab built a promising small-scale computer using this method and completed algorithms using six qubits at a time. Now, they’re working on translating this technology into a larger quantum computer.
It took 300,000 hours of [classical] computing time to solve how three atoms moved. We have to have some kind of alternate approach to work on these problems with a greater dimensionality.
“We see a lot of potential to scale this technology up,” he says.
When Saffman began working in quantum physics in 2001, they could only trap a single atom with a laser.
“We started with very limited knowledge,” he says. His scientific discoveries demonstrate just how much progress has been made in the last two decades.
Saffman is the director of the Wisconsin Quantum Institute, which supports interdisciplinary research across the most promising platforms for scalable quantum computing—and aims to solve problems of the physical world using the methods of quantum science and technology.
“This field has really evolved beyond physics because we’re now at the stage where we know that the fundamental principles and ideas are valid, but we need to actually build full-scale quantum computers,” Saffman explains. “And that involves a lot of engineering, computer science and interdisciplinary work, which the Wisconsin Quantum Institute seeks to coordinate and support.”
Micheline Soley, assistant professor of chemistry and affiliate of the physics department, is one of the researchers affiliated with the institute. Her research sits at the intersection of chemistry, physics, applied mathematics and computer sciences. Her work focuses on studying the ultracold chemistry involved with certain quantum computing methods and developing quantum computing algorithms, which will enable quantum computers to be used for more powerful processing.
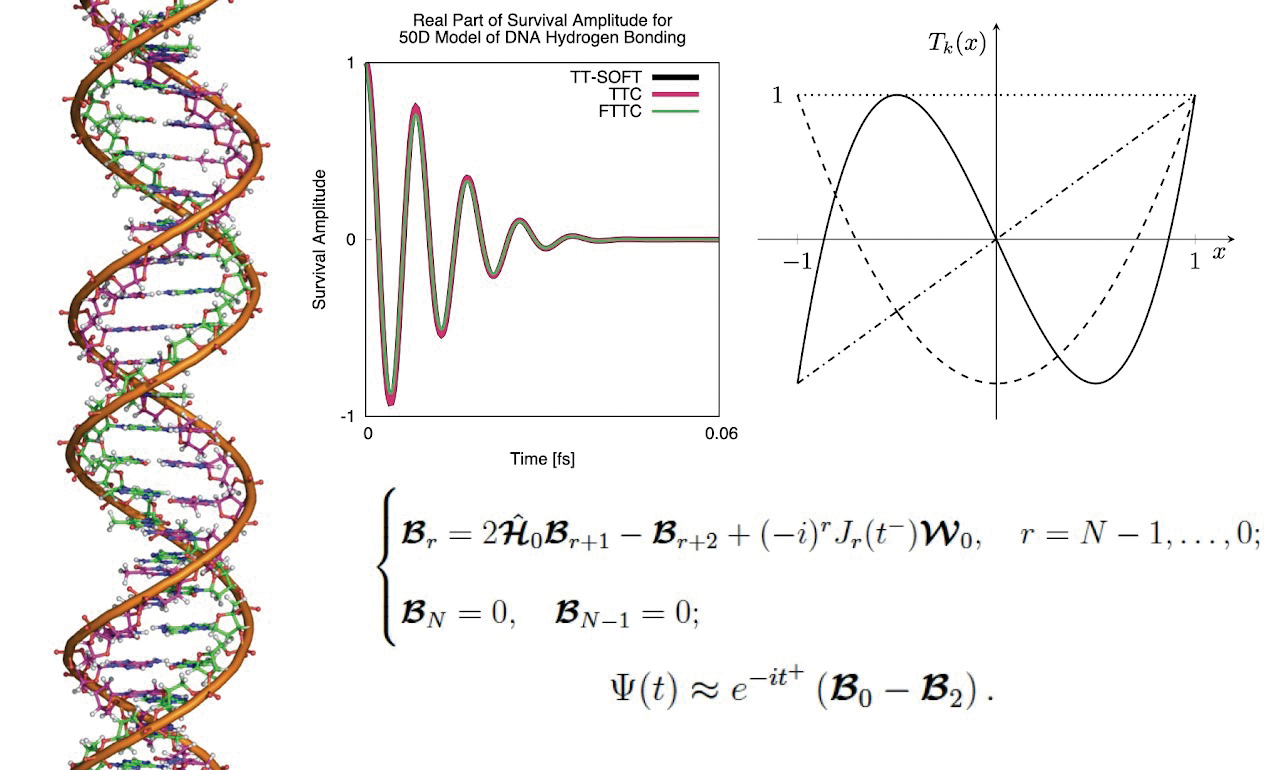
“Quantum computing systems will expand to be able to solve bigger problems that we can’t currently solve, for applications in fields such as energy sciences or pharmaceuticals,” she says.
She points to the example of a classical computer trying to figure out how atoms move through the largest ultracold chemical system whose quantum
movements have been studied exactly.
“It took 300,000 hours of computing time to solve how three atoms moved,” she says. “We have to have some kind of alternate approach to work on these problems with a greater dimensionality.”
Soley is examining how to create quantum methods that would address what’s known as the “curse of dimensionality,” which refers to how the cost of simulating chemical systems grows exponentially with the dimensionality of the chemical system.
Chemistry is a relatively new addition to the quantum field, and Soley is excited about the cyclical potential of quantum computing and chemistry—which is exhibited through her own research. She’s exploring the theory of how to use molecules to build quantum computers and how to use quantum computers to simulate different kinds of molecular systems to solve those bigger problems that classical computers just can’t solve.
The basic science behind quantum computers is exciting, she says, as researchers work toward the common goal of transforming the world’s computers. But, there’s still so much important foundational work that needs to happen to get there, like the science that’s happening across UW.
“This field feels like applied science in so many ways, because we’re building quantum computers and working with them, but on the other end, it’s basic science and it’s still an open field, with so many questions to work on to build really powerful quantum computers. It’s exciting to see the progress and developments that scientists are making.”